Tunable Material Properties
Our group explores the concept of electronically tuneable properties which extends the field-effect principle and reversible electrochemistry to modify a broad spectrum of physical properties in material systems. We are particularly concentrated on field-effect and electrochemically based nanodevices with reversible modulation of various macroscopic properties like electrical, magnetic, mechanical, optical etc. To bring about a surface charge we will make use of electrolyte (liquid and solid), ferroelectrics, and high dielectric constant materials. The general thrust of our research is to establish the scientific foundations enabling the design, synthesis, testing and reversible control of a broad spectrum of nanomaterials and then explore their potential for applications.
Group Leader
Tunable Properties
+49 721 608-25916
robert kruk ∂does-not-exist.kit edu
Radioactive and X-Ray Radiation Safety Officer
Understanding the Magnetoelectric Effect
Controlling Material Surfaces by Electrical Stimuli
Using low-power electric fields to control magnetism (magnetoelectric effect -ME) instead of dissipative flowing currents is considered as a next generation technology to replace conventional methods of data storage and processing, sensing, and actuation. A promising strategy for realization of ME relies on the utilization of composites to couple electric and magnetic polarization at the interface of two functional materials.
The voltage-driven stimulus can be provided by various means, e.g. dielectric, ferroelectric, and electrolyte gating which induces an electric charge at the interface of the functional material leading to charge carrier doping (electric field effect) and thus to a reversibly tuned property change. However going beyond that concept, reversible ion intercalation (reversible electrochemistry), which includes ion transfer across the interface can also effectively alter the materials property. In between these two mechanism, reversible surface chemistry (redox pseudocapacitance), constitutes an effective tools to obtain sizeable surface charges. The different tuning approaches employed in the Tunables group are illustrated schematically in the figure below.
Concepts of tunability in materials
Working Principles of Magnetoelectric Effect (ME) Effect in Artificial Composites
Since in the composite structures the ME effect is of interfacial origin, an understanding of the charging processes that take place at interfaces—or propagate through them—is of crucial importance. The mechanisms acting as mediator of the ME coupling in artificial composites can be divided into three main groups:
a) Mediators of the ME effect in ME composite systems. b) Typical affected length scales in a magnetic material via voltage-driven strain, charge carrier doping, and chemical intercalation. (b) Adapted with permission. Copyright 2015, Wiley
- The first one makes use of the stress induced by a piezoelectric actuator to modify the strain state of a magnetic material, and in turn also its magnetic response via magnetostriction.
- The second, which follows the working principle of the field-effect transistor, is based on the accumulation/depletion of charge carriers in a magnet by the polarization of a gate material.
- The third one relies on the insertion/removal of ions into/from the lattice of a host magnet by using an ionic conductor, analogous to the charging/dis-charging processes occurring in electrochemical batteries.
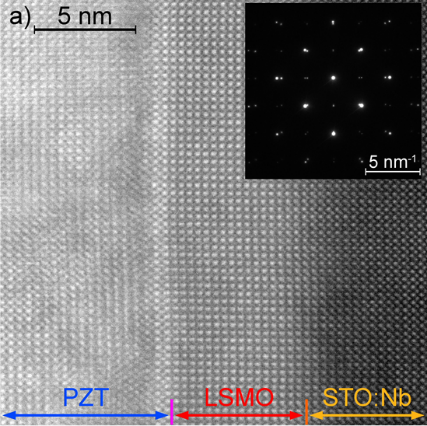
Magnetoelectric coupling at at ferromagnetic/ferroelectric interfaces in epitaxial lanthanium strontium manganite/lead zirconate titanate (LSMO/PZT) heterostructures was studied. The remarkably high lateral uniformity achieved in such films allowed for a ferroelectric device area of more than 6 square milimetres. This has enabled for superconductive quantum interference device (SQUID) measurements of the magnetic response to the systematically, completely in situ, varied remanent ferroelectric polarization. Temperature dependence of the magnetic modulation upon charging and the magnetic response to the ferroelectric stimulation indicate a field-effect dominated coupling mechanism and generally confirm the concept of electrostatic hole doping of LSMO.
Physical Review B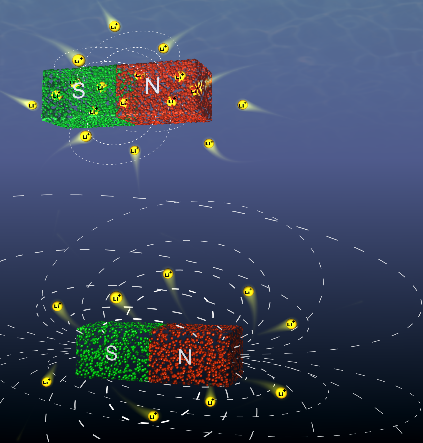
Going beyond the limitation of effects to the material surfaces in field-effect driven systems, we introduce electrochemical intercalation as mean to change magnetism in bulk materials. By inserting Li into a ferromagnetic oxide, we changed the partial distribution of Fe(III) in the spinel structure and show a fully reversible change in magnetism of up to 30 % at room temperature. Considering the vast amount of intercalation-friendly magnetic materials, we see this as the first paving towards complete on-and-off magnetic switching for micro-magnetic actuators.
ADVANCED MATERIALS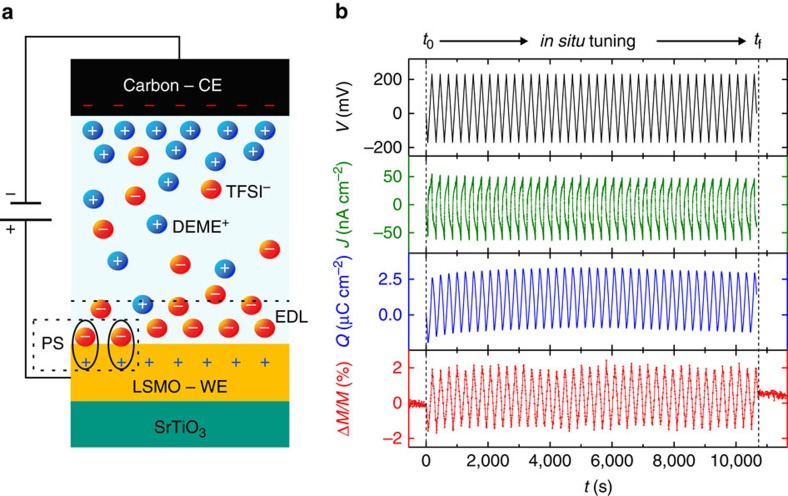
We developed a novel route to robustly tune magnetism using hybrid supercapacitors, i.e. capacitors that combine electrostatic and electrochemical surface charging. Both mechanisms occur at the lanthanium strontium manganite(LSMO)/ionic liquid interface to control the balance between ferromagnetic and non-ferromagnetic phases of LSMO to an unprecedented extent. Magnetic modulation of up to 33 % at only 2.0 V is reached above room temperature. We could discover a new class of reversible physico-chemical phenomena to control magnetism in supercapacitors.
nature communicationsPublications
Singh, S. P.; Witte, R.; Clemens, O.; Sarkar, A.; Velasco, L.; Kruk, R.; Hahn, H.
2020. ACS applied nano materials, 3 (7), 7281–7290. doi:10.1021/acsanm.0c01674
Baksi, A.; Nandam, S. H.; Wang, D.; Kruk, R.; Chellali, M. R.; Ivanisenko, J.; Gallino, I.; Hahn, H.; Bag, S.
2020. ACS applied nano materials, 3 (7), 7252–7259. doi:10.1021/acsanm.0c01584
Witte, R.; Sarkar, A.; Velasco, L.; Kruk, R.; Brand, R. A.; Eggert, B.; Ollefs, K.; Weschke, E.; Wende, H.; Hahn, H.
2020. Journal of applied physics, 127 (18), Art. Nr.: 185109. doi:10.1063/5.0004125
Sarkar, A.; Eggert, B.; Velasco, L.; Mu, X.; Lill, J.; Ollefs, K.; Bhattacharya, S. S.; Wende, H.; Kruk, R.; Brand, R. A.; Hahn, H.
2020. APL materials, 8 (5), Art.Nr. 051111. doi:10.1063/5.0007944
Molinari, A.; Witte, R.; Neelisetty, K. K.; Gorji, S.; Kübel, C.; Münch, I.; Wöhler, F.; Hahn, L.; Hengsbach, S.; Bade, K.; Hahn, H.; Kruk, R.
2020. Advanced materials, 32 (12), Art.-Nr. 1907541. doi:10.1002/adma.201907541
Gack, N.; Iankevich, G.; Benel, C.; Kruk, R.; Wang, D.; Hahn, H.; Reisinger, T.
2020. Nanomaterials, 10 (11), Article: 2192. doi:10.3390/nano10112192
Wang, J.; Cui, Y.; Wang, Q.; Wang, K.; Huang, X.; Stenzel, D.; Sarkar, A.; Azmi, R.; Bergfeldt, T.; Bhattacharya, S. S.; Kruk, R.; Hahn, H.; Schweidler, S.; Brezesinski, T.; Breitung, B.
2020. Scientific reports, 10, Art.-Nr.: 18430. doi:10.1038/s41598-020-75134-1
Baksi, A.; Bag, S.; Kruk, R.; Nandam, S. H.; Hahn, H.
2020. Scientific reports, 10 (1), Art.-Nr.: 17467. doi:10.1038/s41598-020-74507-w
Ye, X.; Singh, H. K.; Zhang, H.; Geßwein, H.; Chellali, M. R.; Witte, R.; Molinari, A.; Skokov, K.; Gutfleisch, O.; Hahn, H.; Kruk, R.
2020. Nature Communications, 11 (1), Art.-Nr.: 4849. doi:10.1038/s41467-020-18552-z
Gack, N.; Reitz, C.; Hemmerich, J. L.; Könne, M.; Bennett, R.; Fiedler, J.; Gleiter, H.; Buhmann, S. Y.; Hahn, H.; Reisinger, T.
2020. Physical review letters, 125 (5), Art.-Nr.: 050401. doi:10.1103/PhysRevLett.125.050401
Neudeck, S.; Mazilkin, A.; Reitz, C.; Hartmann, P.; Janek, J.; Brezesinski, T.
2019. Scientific reports, 9 (1), 5328. doi:10.1038/s41598-019-41767-0
Reitz, C.; Smarsly, B.; Brezesinski, T.
2019. ACS applied nano materials, 2 (2), 1063–1071. doi:10.1021/acsanm.8b02327
Giambra, M. A.; Benfante, A.; Pernice, R.; Miseikis, V.; Fabbri, F.; Reitz, C.; Pernice, W. H. P.; Krupke, R.; Calandra, E.; Stivala, S.; Busacca, A. C.; Danneau, R.
2019. ACS omega, 4 (1), 2256–2260. doi:10.1021/acsomega.8b02836
Androš Dubraja, L.; Kruk, R.; Brezesinski, T.
2019. Advanced electronic materials, 5 (1), Art. Nr.: 1800287. doi:10.1002/aelm.201800287
Dubraja, L. A.; Boll, D.; Reitz, C.; Wang, D.; Belić, D.; Mazilkin, A.; Breitung, B.; Hahn, H.; Elm, M. T.; Brezesinski, T.
2019. ACS applied nano materials, 2 (11), 7126–7133. doi:10.1021/acsanm.9b01654
Singh, R.; Witte, R.; Mu, X.; Brezesinski, T.; Hahn, H.; Kruk, R.; Breitung, B.
2019. Journal of materials chemistry / A, 7 (41), 24005–24011. doi:10.1039/c9ta08928d
Witte, R.; Kruk, R.; Wang, D.; Schlabach, S.; Brand, R. A.; Gruner, M. E.; Wende, H.; Hahn, H.
2019. Physical review / B, 99 (13), Article: 134109. doi:10.1103/PhysRevB.99.134109
Molinari, A.; Hahn, H.; Kruk, R.
2019. Advanced materials, 31 (26), Article: 1806662. doi:10.1002/adma.201806662
Witte, R.; Sarkar, A.; Kruk, R.; Eggert, B.; Brand, R. A.; Wende, H.; Hahn, H.
2019. Physical review materials, 3 (3), Article No.034406. doi:10.1103/PhysRevMaterials.3.034406
Molinari, A.; Hahn, H.; Kruk, R.
2018. Advanced materials, 30 (1), Art.Nr. 1703908. doi:10.1002/adma.201703908
Dräger, C.; Sigel, F.; Witte, R.; Kruk, R.; Pfaffmann, L.; Mangold, S.; Mereacre, V.; Knapp, M.; Ehrenberg, H.; Indris, S.
2018. Physical chemistry, chemical physics. doi:10.1039/C8CP06177G
Dubraja, L. A.; Reitz, C.; Velasco, L.; Witte, R.; Kruk, R.; Hahn, H.; Brezesinski, T.
2018. ACS Applied Nano Materials, 1 (1), 65–72. doi:10.1021/acsanm.7b00037
Benes, A.; Molinari, A.; Witte, R.; Kruk, R.; Brötz, J.; Chellali, R.; Hahn, H.; Clemens, O.
2018. Materials, 11 (1), Art. Nr.: 52. doi:10.3390/ma11010052